Jan Nolta and her colleagues at the Stem Cell Program and Institute for Regenerative Cures at UC Davis have published a remarkable paper in the journal Molecular Therapy regarding Huntington’s disease and a potential stem cell-based strategy to delay the ravages of this disease.
Huntington’s disease (HD) is an inherited neurodegenerative disease. It is inherited as an autosomal dominant disease, which means that someone need only inherit one copy of the disease-causing allele of the HTT gene to have this disease. HD is characterized by progressive cell death in the brain, particularly in a portion of the brain known as the striatum and by widespread brain atrophy.
The portion of the brain known as the striatum lies underneath the surface of the forebrain (subcortical) and it receives neural inputs from the cerebral cortex and is the primary source of neural inputs to the basal ganglia system. The basal ganglia system (BGS) is located underneath the surface of the brain but even deeper within the cerebral hemispheres. The BGS is part of the corpus striatum, it consists of the subthalamic nucleus and the substantia nigra. The BGS help with voluntary motor control, procedural learning relating to routine behaviors. otherwise known as “habits,” eye movements, and cognitive, and emotional functions. The ventral striatum is very important in addiction because it is the reward center on consists of the nucleus accumbens, olfactory tubercle, and islands of Calleja.
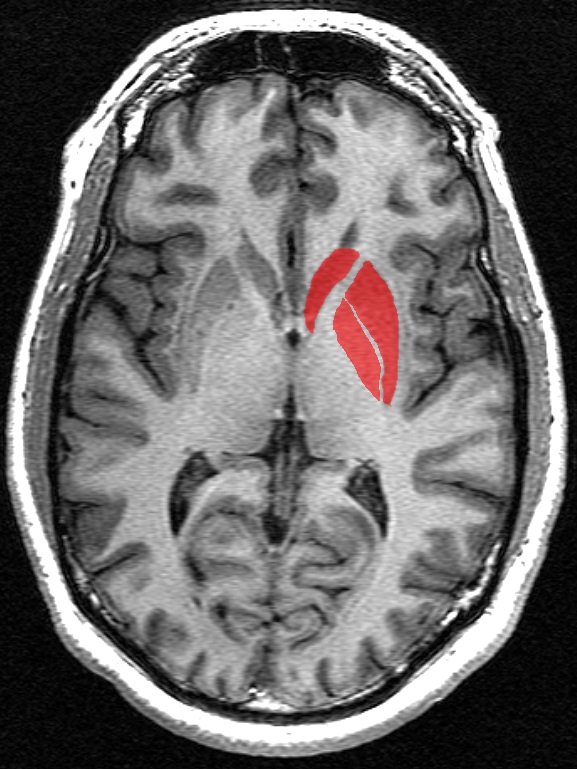
HD takes its largest toll on the striatum, which affects voluntary movement, routine behaviors, and personality. Disturbances of both involuntary and voluntary movements occur in individuals with HD. Chorea, an involuntary movement disorder consisting of nonrepetitive, non-periodic jerking of limbs, face, or trunk, is the major sign of the disease. Chorea is present in more than 90% of individuals, increasing during the first ten years. The choreic movements are continuously present during waking hours, cannot be suppressed voluntarily, and are worsened by stress. HD patients show impaired voluntary motor function early on and show a clumsiness in common daily activities.
With advancing disease duration, other involuntary movements such as slowness of movement (bradykinesia), rigidity, and involuntary muscle contractions that cause repetitive or twisting movements (dystonia) occur. Eye movement becomes progressively worse. So-called “gaze fixation” is observed in ~75% of symptomatic individuals. Unclear speech occurs early and Swallowing difficulties occur later.
Animal models of HD used in the past have injected molecules into the brain that kill off striatal cells and mimic at least some of the characteristics of HD in laboratory animals. Unfortunately, such a model system is fat too clean, since implanted cells tend to survive perfectly well. However the brains of HD patients are like unto a toxic waste dumps and implanted cells are quickly killed off. Therefore, a better animal model system was required, and it came in the form of R6/2 and YAC128 mice. R6/2 mice have a part of the human HTT gene that has 150 CAG triplets, and show the characteristic cell death in the striatum and behavioral deficits. The only problem with this mouse strain is that the neurodegenerative decline is very rapid rather than slow and progressive. YAC128 mice have a full-length copy of the HTT gene and show a slower, more progressive neurological decline that more closely approximates the human clinical condition.
In this paper from the Nolta laboratory, they used a growth factor that is known to decrease precipitously in HD brains; a growth factor called Brain-Derived Neurotrophic Factor (BDNF). BDNF is known to mediate the survival and function of striatal neurons and the reduction of BDNF in the brains of HD patients correlates with the onset of symptoms and the greater the reduction in BDNF, the greater the severity of the disease (see Her LS & Goldstein LS, J Neurosci 2008; 28, 13662-13676).
However injecting BDNF into the brain is problematic, since the protein has a very short half-life. Delivering the growth factor by means of genetically engineered viruses shows promise, but most of the viral vectors used in such experiments are recognized by the immune system as foreign invaders. Therefore, Nolta and her colleagues decided to genetically engineer mesenchymal stem cells (MSCs) to overexpress BDNF and implant these cells into the brains of R6/2 and YAC128 mice.
MSCs have an added advantage over viral vectors: these cells migrate to damaged areas where they can exert their healing properties (see Olson SD et al., Mol Neurobiol 45; 2012: 87-98).
Nolta and her coworkers actually tested human MSCs in HD model mice. After completing all the necessary control experiments to ensure that their isolated and engineered MSCs were secreting BDNF, Nolta and others implanted them into the brains of R6/2 and YAC128 mice.
HD mice show greater anxiety, which is manifested in a so-called “open field assay” by not remaining the center of the field. The control HD mice did not stay long in the center of the open field, but the normal mice did. The MSC-BDNF-implanted mice spend far more time in the center of the field. Mind you, not as much as wild-type mice, but significantly more than their HD counterparts.
Next the volume of the striata of these mice were determined and compared to the normal mice. While all the HD mice showed shrinking of the striatum, the MSC-NDNF-implanted YAC128 mice show significantly less shrinking of the striatum. Then the degree of neurogenesis (formation of new neurons) was measured in normal, HD, HD + implanted MSCs, and HS + MSC-BDNF mice. This experiment measures the degree of healing that is occurring in the brain. The brain from HD + MSC and HD + MSC-BDNF mice showed significantly more new brain cell growth. This is probably the reason for the delayed onset of symptoms and the delayed shrinking of the striatum.
Finally, Nolta and others measured the lifespans of the R6/2 mice and compared them with R6/2 mice that had been implanted with MSCs-BDNF. Animals transplanted with the MSCs that made the most BDNF lived 15% longer than the nontreated R6/2 mice.
MSCs have been shown in several experiments to promote neuronal growth, decrease cell death and decrease inflammation through the secretion of trophic factors. MSCs can modify the toxic environment that is part of the brain of an HD patient and help damaged tissue out by inducing neural regeneration and protection (see Crigler L, et al., Experimental neurology, 198; 2–6, 54-64; Kassis I, et al., Archives of Neurology 65; 2008: 753-761).
The downside of using MSCs that they will only survive in the brain for a few months. However, several studies have shown that the benefits of modified MSC implantation persist after the MSCs are gone, since the neural reconstruction wrought by the secreted BDNF stay after the MSCs have died off (see Arregui L, et al., Cell Mol Neurobiol 31; 2011: 1229-1243 and many others).
At best this treatment would delay the ravages of HD, but delaying this disease might very well be the first step towards a cure. Hopefully, clinical trials will not be fat behind.